(This is a pre-print of my May ‘Cool. Fuel.’ article for Cold Facts — the magazine of the Cryogenic Society of America.)
Back when I was a Ph.D student at Wisconsin the machine that saved many of my days was a Bridgeport manual end mill conveniently located in the basement of the lab. So when I setup the HYPER lab’s manufacturing space at Washington State I found an old Bridgeport clone to place in our manufacturing area. Yes it’s even older now. While it’s still used some for drilling and tapping operations, a new additive manufacturing project led by Jordan Raymond in my lab is making me wonder whether my mill’s years are finally numbered.
Jordan’s masters thesis is on the optimization of a heat exchanger for liquefying hydrogen via a dual stage Gifford-McMahon cooler. Like any good engineer she started with the minimum viable heat exchanger; in this case a copper coiled tube indium-soldered to aluminum blocks bolted to each of the cooler stages. Our pro machine shop used a ball-end mill and a rotary table to machine helical grooves for the coil to nest in (shown in the figure below). We were all impressed they were able to do it. The specs on the finished heat exchanger: 3.15 kg of aluminum, 3.7 m of tube, $4,200, 2.3462 W/K of entropy generated. It worked too. But how much better could we really make it?
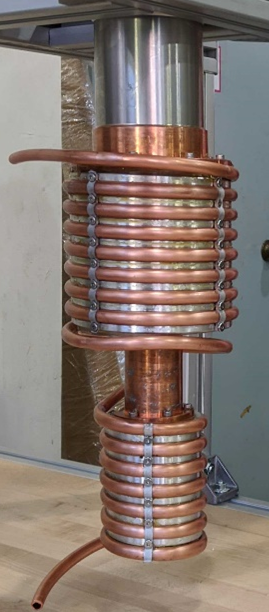
Although I’d designed many heat exchangers using full numerical and sub-compact heat exchanger techniques (I highly recommend Greg Nellis Heat Transfer textbook), I’d never considered designing a heat exchanger from purely entropy generation principles. In short, anytime energy flows through a gradient of some form entropy will be generated. Let’s consider how entropy is generated for flows cooled within a tube:
- With very long and narrow tubes, a pressure gradient to push the fluid through the tube contributes to viscous heating and entropy generation. Circular tubes have the least surface area to contribute to these friction losses.
- With very short and fat tubes, heat must be transferred through considerable temperature gradients between the center of the tube and the wall, resulting in entropy generation. Circular tubes minimize the distance to the centerline, and thereby the temperature difference between the center of the tube and the wall to minimize heat transfer losses.
Whether the flow is laminar or turbulent makes some differences but the two rules above remain. Plotting these generation mechanisms versus length for a fixed tube diameter leads to an interesting optimization problem. Jordan created the following graph for a tube of 1.27 cm inner diameter with a 200 K hydrogen flow at 0.093 g/s. You can see that both of the entropy generation mechanisms are plotted with a minimum total entropy generation appearing near 13 cm in length. This means that for a fixed tube diameter, going any longer or shorter than this length with result in decreased heat exchanger performance. So is that it? Are we stuck? What happens next?
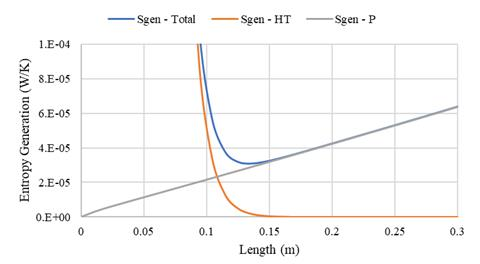
Adrian Bejan figured out that nature found a way around this optimal aspect ratio problem by branching the tube at the optimal length. Splitting the mass flow rate in two leads to a new optimum length to diameter ratio, although both are correspondingly smaller. These branches can then branch again and again, until some other physical limit is approached. Bejan used this entropy branching to predict the shapes of cardiovascular systems in animals, trees, and river deltas among other examples in the physical world. The analogy I enjoy teaching my students comes from the African Savanna. Giraffes, with their long and thin necks, run into pressure based entropy generation limitations and evolved hearts that crank out 300/180 mmHg blood pressure. Elephants, with their bulbous bodies and thick limbs, run into temperature based entropy generation limitations and evolved ear flaps for enhanced cooling. That’s all fine, but I always wondered how any of this would apply to cryogenic hardware.
Selective Laser Sintering (SLS) is a type of additive manufacturing that grows polymer or metallic parts from a powder bed. Jordan found a printer capable of producing aluminum alloys that could print the branching networks with nodes for attaching to the cooler stages. Jordan was even able to vary the wall thickness along the branch lengths to account for the increasing heat transfer the walls would accumulate from the fluid. Although the properties of the printable alloys have yet to be characterized for cryogenics, we are setting the systems up to do those measurements. The figure below shows her ‘grown’ or printed heat exchanger. The specs on the printed heat exchanger were interesting: 2.20 kg of aluminum, 0.3185 m of tube, $8,633, 1.3973 W/K of entropy generated. She was even able to put bends in the tubes to account for thermal contraction relative to the cooler. Although comparably more expensive, the performance specifications are far superior.
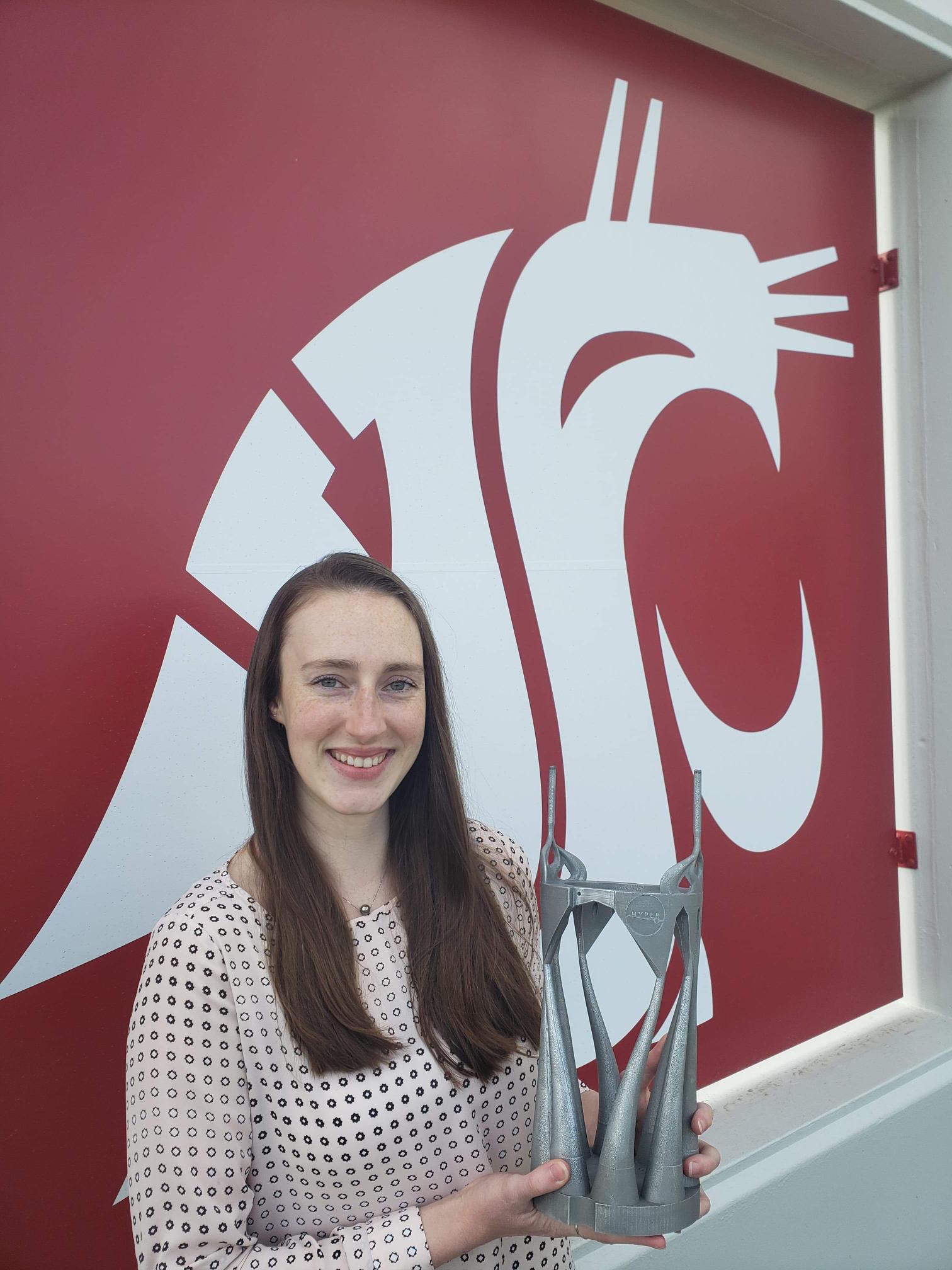
Given the improved metrics, I’m looking forward to a future where we can grow more optimal parts for use in cryogenics. Most of my students have a 3D printer of some kind in their apartments now and are comfortable thinking additively rather than reductively. So it looks like additive manufacturing is one area where we can count on future growth.